Abstract
Heavy-fermion systems represent one of the paradigmatic strongly correlated states of matter1,2,3,4,5. They have been used as a platform for investigating exotic behaviour ranging from quantum criticality and non-Fermi liquid behaviour to unconventional topological superconductivity4,5,6,7,8,9,10,11,12. The heavy-fermion phenomenon arises from the exchange interaction between localized magnetic moments and conduction electrons leading to Kondo lattice physics, and represents one of the long-standing open problems in quantum materials3. In a Kondo lattice, the exchange interaction gives rise to a band with heavy effective mass. This intriguing phenomenology has so far been realized only in compounds containing rare-earth elements with 4f or 5f electrons1,4,13,14. Here we realize a designer van der Waals heterostructure where artificial heavy fermions emerge from the Kondo coupling between a lattice of localized magnetic moments and itinerant electrons in a 1T/1H-TaS2 heterostructure. We study the heterostructure using scanning tunnelling microscopy and spectroscopy and show that depending on the stacking order of the monolayers, we can reveal either the localized magnetic moments and the associated Kondo effect, or the conduction electrons with a heavy-fermion hybridization gap. Our experiments realize an ultimately tunable platform for future experiments probing enhanced many-body correlations, dimensional tuning of quantum criticality and unconventional superconductivity in two-dimensional artificial heavy-fermion systems15,16,17.
Access options
Subscribe to Journal
Get full journal access for 1 year
199,00 €
only 3,90 € per issue
Tax calculation will be finalised during checkout.
Rent or Buy article
Get time limited or full article access on ReadCube.
from$8.99
All prices are NET prices.
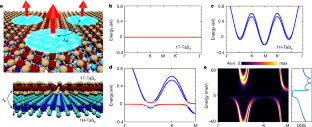
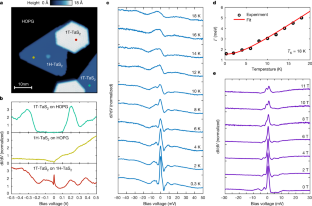
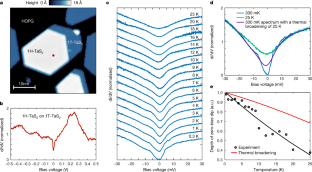
Data availability
All of the data supporting the findings are available from the corresponding authors upon request.
References
- 1.
Stewart, G. R. Heavy-fermion systems. Rev. Mod. Phys. 56, 755–787 (1984).
- 2.
Yazdani, A., da Silva Neto, E. H. & Aynajian, P. Spectroscopic imaging of strongly correlated electronic states. Annu. Rev. Condens. Matter Phys. 7, 11–33 (2016).
- 3.
Dzero, M., Xia, J., Galitski, V. & Coleman, P. Topological Kondo insulators. Annu. Rev. Condens. Matter Phys.7, 249–280 (2016).
- 4.
Wirth, S. & Steglich, F. Exploring heavy fermions from macroscopic to microscopic length scales. Nat. Rev. Mater. 1, 16051 (2016).
- 5.
Jiao, L. et al. Chiral superconductivity in heavy-fermion metal UTe2. Nature 579, 523–527 (2020).
- 6.
Pfleiderer, C. et al. Partial order in the non-Fermi-liquid phase of MnSi. Nature 427, 227–231 (2004).
- 7.
Stewart, G. R. Non-Fermi-liquid behavior in d- and f-electron metals. Rev. Mod. Phys. 73, 797–855 (2001).
- 8.
Löhneysen, H. V., Rosch, A., Vojta, M. & Wölfle, P. Fermi-liquid instabilities at magnetic quantum phase transitions. Rev. Mod. Phys. 79, 1015–1075 (2007).
- 9.
Gegenwart, P., Si, Q. & Steglich, F. Quantum criticality in heavy-fermion metals. Nat. Phys. 4, 186–197 (2008).
- 10.
Aynajian, P. et al. Visualizing heavy fermions emerging in a quantum critical Kondo lattice. Nature 486, 201–206 (2012).
- 11.
Allan, M. P. et al. Imaging Cooper pairing of heavy fermions in CeCoIn5. Nat. Phys. 9, 468–473 (2013).
- 12.
Zhou, B. B. et al. Visualizing nodal heavy fermion superconductivity in CeCoIn5. Nat. Phys. 9, 474–479 (2013).
- 13.
Chen, C., Sodemann, I. & Lee, P. A. Competition of spinon Fermi surface and heavy Fermi liquid states from the periodic Anderson to the Hubbard model. Phys. Rev. B 103, 085128 (2021).
- 14.
Ramires, A. & Lado, J. L. Emulating heavy fermions in twisted trilayer graphene. Phys. Rev. Lett. 127, 026401 (2021).
- 15.
Neumann, M., Nyéki, J., Cowan, B. & Saunders, J. Bilayer 3He: a simple two-dimensional heavy-fermion system with quantum criticality. Science 317, 1356–1359 (2007).
- 16.
Shishido, H. et al. Tuning the dimensionality of the heavy fermion compound CeIn3. Science 327, 980–983 (2010).
- 17.
Mizukami, Y. et al. Extremely strong-coupling superconductivity in artificial two-dimensional Kondo lattices. Nat. Phys. 7, 849–853 (2011).
- 18.
Geim, A. K. & Grigorieva, I. V. Van der Waals heterostructures. Nature 499, 419–425 (2013).
- 19.
Liu, Y. et al. Van der Waals heterostructures and devices. Nat. Rev. Mater. 1, 16042 (2016).
- 20.
Novoselov, K. S., Mishchenko, A., Carvalho, A. & Castro Neto, A. H. 2D materials and van der Waals heterostructures. Science 353, aac9439 (2016).
- 21.
Kennes, D. M. et al. Moiré heterostructures as a condensed-matter quantum simulator. Nat. Phys. 17, 155–163 (2021).
- 22.
Andrei, E. Y. et al. The marvels of moiré materials. Nat. Rev. Mater. 6, 201–206 (2021).
- 23.
Ugeda, M. M. et al. Characterization of collective ground states in single-layer NbSe2. Nat. Phys. 12, 92–97 (2015).
- 24.
de la Barrera, S. C. et al. Tuning Ising superconductivity with layer and spin–orbit coupling in two-dimensional transition-metal dichalcogenides. Nat. Commun. 9, 1427 (2018).
- 25.
Law, K. T. & Lee, P. A. 1T-TaS2 as a quantum spin liquid. Proc. Natl Acad. Sci. USA 114, 6996–7000 (2017).
- 26.
Cho, D. et al. Nanoscale manipulation of the Mott insulating state coupled to charge order in 1T-TaS2. Nat. Commun. 7, 10453 (2016).
- 27.
Qiao, S. et al. Mottness collapse in 1T-TaS2−xSex transition-metal dichalcogenide: an interplay between localized and itinerant orbitals. Phys. Rev. X 7, 041054 (2017).
- 28.
Chen, Y. et al. Strong correlations and orbital texture in single-layer 1T-TaSe2. Nat. Phys. 16, 218–224 (2020).
- 29.
Ruan, W. et al. Evidence for quantum spin liquid behaviour in single-layer 1T-TaSe2 from scanning tunnelling microscopy. Nat. Phys. 17, 1154–1161 (2021).
- 30.
Kratochvilova, M. et al. The low-temperature highly correlated quantum phase in the charge-density-wave 1T-TaS2 compound. npj Quant. Mater. 2, 42 (2017).
- 31.
Coqblin, B. & Schrieffer, J. R. Exchange interaction in alloys with cerium impurities. Phys. Rev. 185, 847–853 (1969).
- 32.
Wen, C. et al. Roles of the narrow electronic band near the Fermi level in 1T-TaS2-related layered materials. Phys. Rev. Lett. 126, 256402 (2021).
- 33.
Zhang, Y.-H. et al. Temperature and magnetic field dependence of a Kondo system in the weak coupling regime. Nat. Commun. 4, 2110 (2013).
- 34.
Ryu, H. et al. Persistent charge-density-wave order in single-layer TaSe2. Nano Lett. 18, 689–694 (2018).
- 35.
McMillan, W. L. & Mochel, J. Electron tunneling experiments on amorphous Ge1−xAux. Phys. Rev. Lett. 46, 556–557 (1981).
- 36.
Ernst, S. et al. Emerging local Kondo screening and spatial coherence in the heavy-fermion metal YbRh2Si2. Nature 474, 362–366 (2011).
- 37.
Röβler, S. et al. Hybridization gap and Fano resonance in SmB6. Proc. Natl Acad. Sci. USA 111, 4798–4802 (2014).
- 38.
Bose, S. & Ayyub, P. A review of finite size effects in quasi-zero dimensional superconductors. Rep. Prog. Phys. 77, 116503 (2014).
- 39.
Ganguli, S. C., Vaňo, V., Kezilebieke, S., Lado, J. L. & Liljeroth, P. Controlling correlations in NbSe2 via quantum confinement. Preprint at https://arxiv.org/abs/2009.13422 (2020).
- 40.
Kouwenhoven, L. P., Austing, D. G. & Tarucha, S. Few-electron quantum dots. Rep. Prog. Phys. 64, 701–736 (2001).
- 41.
She, J.-H., Kim, C. H., Fennie, C. J., Lawler, M. J. & Kim, E.-A. Topological superconductivity in metal/quantum-spin-ice heterostructures. npj Quant. Mater. 2, 64 (2017).
- 42.
Ribak, A. et al. Chiral superconductivity in the alternate stacking compound 4Hb-TaS2. Sci. Adv. 6, eaax9480 (2020).
- 43.
Seiro, S. et al. Evolution of the Kondo lattice and non-Fermi liquid excitations in a heavy-fermion metal. Nat. Commun. 9, 3324 (2018).
- 44.
Hall, J. et al. Molecular beam epitaxy of quasi-freestanding transition metal disulphide monolayers on van der Waals substrates: a growth study. 2D Mater. 5, 025005 (2018).
- 45.
Lin, H. et al. Growth of atomically thick transition metal sulfide films on graphene/6H-SiC(0001) by molecular beam epitaxy. Nano Res. 11, 4722–4727 (2018).
Acknowledgements
This research made use of the Aalto Nanomicroscopy Center (Aalto NMC) facilities and was supported by the European Research Council (ERC-2017-AdG no. 788185 “Artificial Designer Materials”), the Academy of Finland (Academy professor funding nos. 318995 and 320555, Academy postdoctoral researcher no. 309975, Academy research fellow nos. 331342 and 336243) and the Jane and Aatos Erkko Foundation. We acknowledge the computational resources provided by the Aalto Science-IT project.
Author information
Affiliations
Contributions
V.V., J.L.L., S.K. and P.L. conceived the experiment, V.V., M.A., S.C.G. and S.K. carried out the sample growth and the low-temperature STM experiments. V.V. analysed the STM data. G.C. and J.L.L. developed the theoretical model. V.V., J.L.L. and P.L. wrote the manuscript with input from all co-authors.
Corresponding authors
Ethics declarations
Competing interests
The authors declare no competing interests.
Additional information
Peer review information Nature thanks Milan Allan, Stefan Kirchner and the other, anonymous, reviewer(s) for their contribution to the peer review of this work. Peer reviewer reports are available.
Publisher’s note Springer Nature remains neutral with regard to jurisdictional claims in published maps and institutional affiliations.
Extended data figures and tables
Extended Data Fig. 1 Grid spectroscopy measurement of a 1T-TaS2/1H-TaS2 heterostructure.
a, STM image of a 1T/1H-TaS2 heterostructure (V = 200 mV and I = 10 pA). b, Tunneling spectra across the red line shown in (a), starting from top left. We observe a Kondo peak located on each of the CDW centers. c, dI/dV map at V = 0 mV measured on the area shown in (a) showing the Kondo lattice. Due to a Kondo peak, there is a higher dI/dV intensity on each of the CDW centers. Middle and bottom rows: dI/dV maps at given bias voltages, all measured on the area shown in (a) and using the same colour scale as in (c). Each of the dI/dV curves was normalized by dividing it by its mean value. Single tunneling spectra on different CDW centers and on a different area are shown in Extended Data Fig. 2.
Extended Data Fig. 2 Single tunneling spectra in the centre of different CDW unit cells.
Left: STM image of a 1T-TaS2/1H-TaS2 heterostructure (V = 200 mV and I = 20 pA). Right: tunneling spectra measured at locations highlighted by the same-coloured dots on the STM image, spectra are vertically offset for clarity.
Extended Data Fig. 3 Fitting of the Kondo resonances.
Left: Temperature dependence of a Kondo resonance in tunneling spectroscopy (black lines), and their respective fits to the Fano lineshape (red lines). The spectra are vertically offset for clarity. Right: Table of the fit parameters.
Extended Data Fig. 4 Thermal broadening of the Kondo resonance.
Tunneling spectroscopy of a Kondo resonance at 300 mK (blue), 18 K (green), and a simulated tunneling spectrum at 18 K (red), where the 300 mK spectrum was taken as the density of states.
Extended Data Fig. 5 Tunneling spectra of 1H-TaS2 on different substrates.
Tunneling spectroscopy of 1H-TaS2 on HOPG (purple line), bilayer 1H-TaS2 (blue line) and 1H-TaS2 on monolayer 1T-TaS2 (green line). The spectra are measured on positions highlighted by the same-coloured dots in the STM image on the left. The spectra exhibit a dip around the Fermi level, but while 1H-TaS2 on HOPG (purple line) and bilayer 1H-TaS2 (blue line) have finite zero-bias conductance, only the spectroscopy of 1H/1T-TaS2 (green line) exhibits a heavy-fermion gap with zero conductance at zero bias.
Extended Data Fig. 6 Examples of different 1H/1T-TaS2 heterostructures.
STM images (left) and corresponding tunneling spectra measured on top of a heterostructure (right). Purple dots highlight the positions, where the tunneling spectra were taken. All the heterostructures exhibit a heavy-fermion gap with zero conductance at zero bias and approximately the same gap width, regardless of the island size.
Extended Data Fig. 7 Tunneling spectroscopy across middle of the 1H/1T-TaS2 island.
a, STM image of a 1H/1T-TaS2 vertical heterostructure (V = 900 mV and I = 20 pA). b, Tunneling spectra across the red line shown in (a). c, Average tunneling spectrum from the spectra across line in (b).
Extended Data Fig. 8 Spatial dependence of the heavy-fermion gap.
a, STM image of a 1H-TaS2/1T-TaS2 heterostructure (V = 50 mV and I = 500 pA). Larger scale topographic image is shown in Extended Data Fig. 9e. b, Tunneling spectra across the red line shown in (a). c, dI/dV map at V = 0 mV measured on the area shown in (a). Middle and bottom rows: dI/dV maps at given bias voltages, all measured on the area shown in (a) and using the same colour scale as in (c).
Extended Data Fig. 9 High-resolution STM images of different heterostructures.
a, c, e, g, Large area STM images. b, STM image of a 1H-TaS2 on HOPG (V = 50 mV and I = 500 pA). Inset shows fast Fourier transform of the image. d, STM image of a monolayer 1T-TaS2 on HOPG (V = 1 V and I = 20 pA). f, STM image of a 1H/1T-TaS2 vertical heterostructure (V = 50 V and I = 500 pA). Inset shows fast Fourier transform of the image. h, STM image of a 1T/1H-TaS2 vertical heterostructure (V = 0.3 V and I = 50 pA). 1H-TaS2 on HOPG exhibits a strong 3×3 CDW, while 1H-TaS2 on 1T-TaS2 shows no signs of CDW. Both 1T-TaS2 on HOPG and 1T-TaS2 on 1H-TaS2 exhibit a strong √13×√13 CDW.
Extended Data Fig. 10 Magnetic field dependence of a heavy-fermion gap.
Tunneling spectroscopy of a heavy-fermion gap measured on a 1H/1T-TaS2 vertical heterostructure at different applied magnetic fields, the spectra are vertically offset for clarity.
Supplementary information
Supplementary Information
This file contains Supplementary Sections 1–3: detailed description of the theoretical model; discussion on the comparison with the magnetic field dependence of natural heavy-fermion compounds; discussion on the potential further probes of the heavy-fermion regime.
Rights and permissions
About this article
Cite this article
Vaňo, V., Amini, M., Ganguli, S.C. et al. Artificial heavy fermions in a van der Waals heterostructure. Nature 599, 582–586 (2021). https://ift.tt/3l5w3Pv
-
Received:
-
Accepted:
-
Published:
-
Issue Date:
Comments
By submitting a comment you agree to abide by our Terms and Community Guidelines. If you find something abusive or that does not comply with our terms or guidelines please flag it as inappropriate.
"heavy" - Google News
November 24, 2021 at 11:13PM
https://ift.tt/3xnZFMP
Artificial heavy fermions in a van der Waals heterostructure - Nature.com
"heavy" - Google News
https://ift.tt/35FbxvS
https://ift.tt/3c3RoCk
heavy
Bagikan Berita Ini
0 Response to "Artificial heavy fermions in a van der Waals heterostructure - Nature.com"
Post a Comment